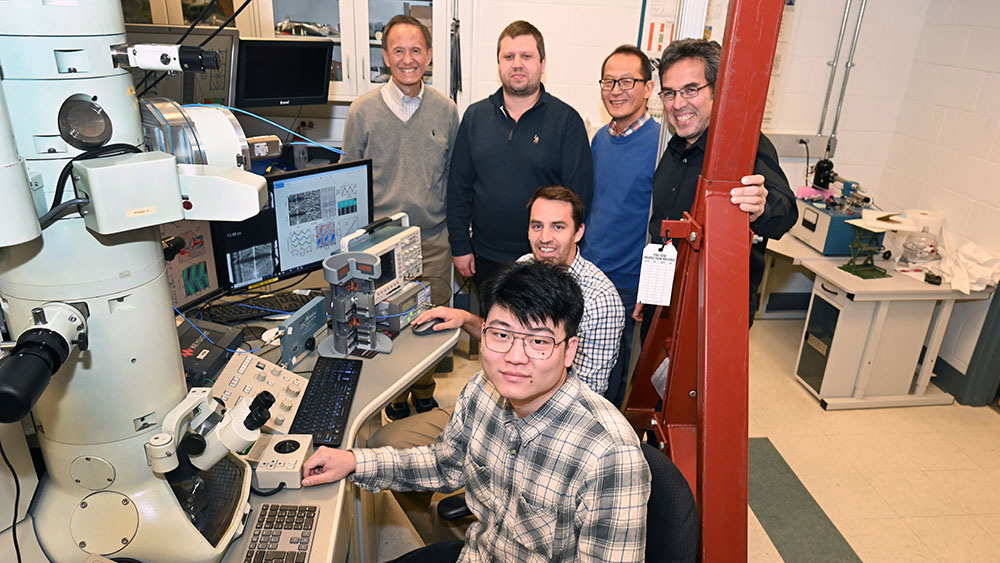
Kevin Coughlin/Brookhaven National Laboratory
Members of the Brookhaven research team next to the Lorentz transmission electron microscope: Yimei Zhu, Alex Pofelski, Myung-Geun Han, and Fernando Camino (back row); Spencer Reisbeck (middle); and Chuhang Liu (front).
One major category of the next generation of energy-efficient microelectronic devices and information processing technologies will likely be based on “spintronics,” which leverages both an electron’s charge and its spin—the tiny “up” or “down” magnetic moment carried by every electron. Now, a relatively new subset of spintronics has sprung up, known as magnonics, which harnesses the collective behaviors of spins, known as spin waves or magnons.
ADVERTISEMENT |
To advance the development of magnonics, researchers still have much to learn about spin waves in magnetic materials. One challenge has been how to most effectively image spin waves at the billionth-of-a-meter level, or nanoscale, because current microscopy techniques are neither sufficiently sensitive to spin nor “fast” enough to actually image magnon behaviors, which take place on extremely short timescales.
Now, however, a group of researchers at the U.S. Department of Energy’s Brookhaven National Laboratory has developed a way to image spin waves in highly compressed real time using an electron microscope coupled with microwave technology. Their work is a significant breakthrough for the fields of electron microscopy and magnonics. It’s described in the Jan. 27, 2025, issue of the journal Nature Materials.
“Our imaging setup is truly innovative, allowing us to directly observe spin-wave behavior with both an unparalleled high spatial and temporal resolution,” says Chuhang Liu, the paper’s lead author, who is a Ph.D. student in the Department of Physics and Astronomy at Stony Brook University and is conducting his thesis research at Brookhaven. “This is the first time that spin waves have been observed with electron microscopy.”
The study opens new frontiers in magnon research and beyond. Developments in “ultrafast” imaging techniques, such as this one, are essential to advancing the field of neuromorphic computing, where researchers try to replicate the energy efficiency and parallel processing capabilities of the human brain while operating at even faster speeds. In the human brain, neurons process and transmit information, while synapses connect neurons and facilitate the transfer of signals through neurotransmitters, enabling learning and memory formation.
“The ultimate objective of our study, and neuromorphic computing in general, is to understand and realize brain-like functionality with artificial systems,” says Liu’s advisor, senior Brookhaven physicist Yimei Zhu, the corresponding author of the paper, who initiated the project.
Spin waves in magnetic materials
Probing spin states and spin waves, and learning how to control them, is vital for the development of modern and future technologies such as energy-efficient computing, advanced memory, and quantum devices. Spintronics and magnonics minimize the energy losses associated with charge currents in traditional devices and enable faster signal processing.
In this work, the team created and stabilized a unique topological magnetic structure in permalloy thin films using lithography patterning and microwave technology. These films contain “spin vortices,” which are localized regions in which the spins curl around in a circular, vortex-like pattern; “anti-vortices” featuring an opposite spin chirality; and various types of magnetic domain walls that connect them.
Through an antenna, the group applied radio frequency electric signals to the sample, causing an excitation of the spins. From there, the group was able to observe the generation, propagation, reflection, and interference of the spin waves. The study revealed that the spin waves preferentially form at anti-vortices, and it also showed that the spin wave emission is associated with an oscillatory motion of specific domain walls. These findings offer valuable insights into the mechanisms of spin wave formation and transmission in magnonic systems, advancing scientists’ understanding of energy-efficient signal processing and how these mechanisms might be used to develop new technologies.
Another electron microscopy first: Imaging spin-wave dynamics
Zhu and his group acquired the United States’ first dedicated Lorentz transmission electron microscope (LTEM) at Brookhaven two decades ago to image spin structures in magnetic materials and thin films. In an LTEM, an electron beam passing through a magnetic sample is reflected due to its interaction with the spins in the sample, generating a Lorentz force. By analyzing the deflections of the electron beam, magnetic structure and spin states and their dynamics can be imaged and studied.
The concept of imaging spin waves was developed at Brookhaven in 2014. The group coupled the LTEM with a microwave-frequency-mediated, ultrafast electron pulser, a device that generates picosecond electron bunches in transmission electron microscopes (TEMs) without the use of pulsed lasers. The pulser was originally developed in partnership with Euclid Techlabs, a small accelerator technology company, under the support of Energy’s Small Business Innovation Research grants. The development earned an R&D 100 Award in 2019 and a Microscopy Innovation Award in 2020, garnering significant attention and recognition in the field of electron microscopy.
However, the ability to capture spin wave dynamics represents a new level of accomplishment, because imaging weak spin signals at a speed of a few picoseconds (trillionths of a second) per frame is extremely challenging.
“This requires the development of advanced hardware and software, for example a single-electron-sensitive ultrafast detection system and complex computational algorithms for precise synchronization of data acquisition and alignment of hundreds of submicron-scale images—a task even more daunting than finding a needle in a haystack,” says Brookhaven physicist Spencer Reisbick, a co-author of the paper.
Through persistent efforts, the group successfully achieved controlled microwave excitation to generate spin waves and capture their dynamic behavior with precision.
The work also highlights the critical importance of electrical triggering for practical applications, because ultrafast research traditionally relies on photoexcitation for fundamental studies. Pure electrical excitation replicates the electric-spike-based signaling found in biological synapses, a feature essential for mimicking neural network behavior.
“Our work opens a new frontier in electron microscopy, offering an unprecedented nanoscale view of magnon dynamics,” says Zhu. “The introduction of microwave imaging in TEM is a significant breakthrough, as modern wireless technologies and quantum qubits operate at gigahertz frequencies. By combining high-frequency electric excitation with imaging, we aim to bridge the gap between fundamental research and practical real-world applications.”
This work was supported by Energy’s Office of Science. The lithography patterning of the films was carried out at the Center for Functional Nanomaterials, an Office of Science user facility at Brookhaven.
Published Feb. 12, 2025, by Brookhaven National Laboratory.
Add new comment